Time to read: 6 minute read
Originally published : Tue, July 4, 2023 @ 4:28 PM
Updated : Fri, July 7, 2023
Over the last few decades, researchers have made impressive strides in developing methods to overcome the delivery, stability, and specificity challenges of oligonucleotide therapeutics. Although we are yet to harness their full potential, the current pace is nothing short of exhilarating.
As of 2023, 18 nucleic acid therapies have made it to the market and many more are in the late clinical development phase.1 Among these, antisense oligonucleotides (ASOs) and small-interfering RNAs (siRNAs) make up a major segment, while other types such as miRNAs, aptamers, and DNAzymes are also under development.2 In this article, we review the latest technical advances to enhance the biological action of ASOs and siRNAs.
As of 2023, 18 nucleic acid therapies have made it to the market and many more are in the late clinical development phase.1 Among these, antisense oligonucleotides (ASOs) and small-interfering RNAs (siRNAs) make up a major segment, while other types such as miRNAs, aptamers, and DNAzymes are also under development.2 In this article, we review the latest technical advances to enhance the biological action of ASOs and siRNAs.
How do ASO and siRNA therapies work?
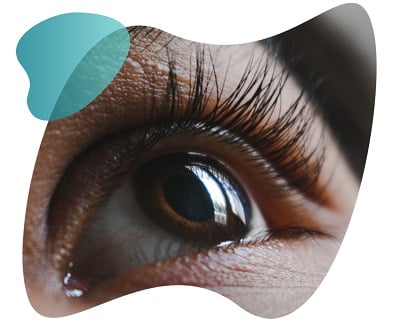
The mechanism of action
ASOs are small, single-stranded oligodeoxyribonucleotides comprising 8-50 bases that can modulate the expression of specific target RNAs via two distinct mechanisms: gene expression inhibition and splicing modulation. Expression inhibitors have a DNA-like structure and form DNA-RNA hetroduplexes that are selectively degraded by the RNAseH enzyme. Whereas splicing modulators bind to the intron-exon junctions and alter splicing events by exon skipping or inclusion.2,3
ASOs are small, single-stranded oligodeoxyribonucleotides comprising 8-50 bases that can modulate the expression of specific target RNAs via two distinct mechanisms: gene expression inhibition and splicing modulation. Expression inhibitors have a DNA-like structure and form DNA-RNA hetroduplexes that are selectively degraded by the RNAseH enzyme. Whereas splicing modulators bind to the intron-exon junctions and alter splicing events by exon skipping or inclusion.2,3
siRNAs are double stranded RNAs of 19-21 bases that work by RNA-interference. Upon cellular uptake, the siRNA gets incorporated into the RNA-induced silencing complex (RISC), where the antisense strand guides the RISC complex to the target mRNA, leading to its degradation.3
The challenges
Unmodified oligonucleotides are susceptible to degradation by endogenous nucleases, have limited cell-membrane permeability and are quickly cleared by the kidneys. To overcome these challenges, clinical development programs have focused on modifying the structure and chemistry of nucleic acid therapeutics to improve their pharmacokinetic properties and clinical efficacy.4
Chemical modifications such as phosphonate modifications, ribose modifications and/or base modifications have been developed for this purpose. In addition, formulations and conjugations with specific chemical groups or receptor ligands have been created to overcome delivery limitations and increase tissue specificity and cell uptake. Some examples of these technologies include lipophilic modifications such as cholesterol and palmitic acid, vitamin-based modifications and N-acetylgalactosamine (GalNAc) conjugates.
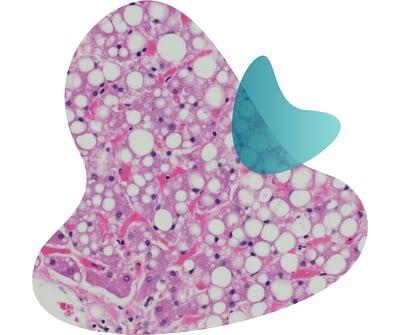
You can learn more about how chemical modifications enable therapeutic applications in our previous article: Overcoming manufacturing challenges associated with nucleic acid therapeutics.
The progression of RNA-targeting therapeutics
Nucleic acid therapeutics are generally categorised into three generations based on the types of modifications they undergo. These include:
First generation
The first generation of oligonucleotide therapeutics featured a phosphorothioate backbone and other modifications, such as methyl-phosphonate, to increase resistance to nuclease degradation. Phosphorothioate oligos are amphipathic, allowing them to bind to proteins in serum, cell surfaces, and intracellularly. These interactions facilitate effective cell uptake and tissue distribution, making it possible to administer phosphorothioate ASOs through various routes in saline.5 However, siRNAs are double-stranded and have a higher molecular weight. These characteristics mean they do not bind to serum proteins and are quickly eliminated from the body. This makes tissue delivery difficult, requiring specialised delivery systems, such as lipid or nanoparticle formulations or chemical modifications with cell-surface receptor binding moieties.3,6 In addition, siRNAs need to be carefully designed to avoid off-target binding as RISC is tolerant to minor mismatches and to prevent the activation of toll-like receptors, which could potentially cause systemic toxicity.7
The first generation of oligonucleotide therapeutics featured a phosphorothioate backbone and other modifications, such as methyl-phosphonate, to increase resistance to nuclease degradation. Phosphorothioate oligos are amphipathic, allowing them to bind to proteins in serum, cell surfaces, and intracellularly. These interactions facilitate effective cell uptake and tissue distribution, making it possible to administer phosphorothioate ASOs through various routes in saline.5 However, siRNAs are double-stranded and have a higher molecular weight. These characteristics mean they do not bind to serum proteins and are quickly eliminated from the body. This makes tissue delivery difficult, requiring specialised delivery systems, such as lipid or nanoparticle formulations or chemical modifications with cell-surface receptor binding moieties.3,6 In addition, siRNAs need to be carefully designed to avoid off-target binding as RISC is tolerant to minor mismatches and to prevent the activation of toll-like receptors, which could potentially cause systemic toxicity.7
Second generation
The next generation of oligonucleotide therapeutics incorporates modifications at the 2′ position of the sugar moiety in addition to a phosphorothioate backbone. These modifications increase nuclease resistance a notch further and also enhance the binding affinity to target RNAs.5 The most commonly used modifications include 2′-O-methyl (2′-OMe), 2′-O-methoxyethyl (2′-MOE), and 2′-fluoro (2′-F).8 Here, it is worth noting that ASOs with such modifications have greater affinity for their target RNAs and lesser cytotoxicity but cannot recruit RNase H1 for RNA cleavage, which is essential for RNaseH-dependent ASOs. To overcome this limitation, the central DNA segment is retained and flanked by nucleotides of modified chemistry, resulting in what is known as gapmers.9
The next generation of oligonucleotide therapeutics incorporates modifications at the 2′ position of the sugar moiety in addition to a phosphorothioate backbone. These modifications increase nuclease resistance a notch further and also enhance the binding affinity to target RNAs.5 The most commonly used modifications include 2′-O-methyl (2′-OMe), 2′-O-methoxyethyl (2′-MOE), and 2′-fluoro (2′-F).8 Here, it is worth noting that ASOs with such modifications have greater affinity for their target RNAs and lesser cytotoxicity but cannot recruit RNase H1 for RNA cleavage, which is essential for RNaseH-dependent ASOs. To overcome this limitation, the central DNA segment is retained and flanked by nucleotides of modified chemistry, resulting in what is known as gapmers.9
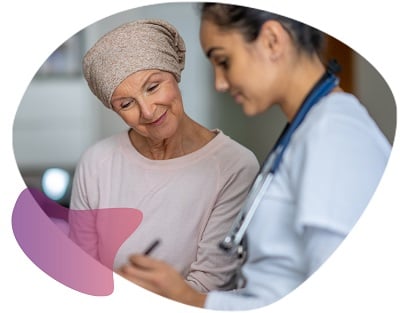
Third generation
The latest generation of oligonucleotide therapeutics features base modifications that offer even greater binding affinity. These include tricyclo-DNA (tcDNA), locked nucleic acid (LNA), peptide nucleic acid (PNA) and phosphorodiamidate morpholino oligomer (PMO). Owing to the high liver toxicity issues of LNAs, constrained methyl- and ethyl-bridges in constrained methoxyethyl (cMOE) and constrained ethyl (cEt) oligonucleotides are often utilised.5 However, PMOs and PNAs are less toxic. But, they are neutrally charged and exhibit poor cellular uptake, requiring a bio-conjugate or carrier for effective delivery.5
The latest generation of oligonucleotide therapeutics features base modifications that offer even greater binding affinity. These include tricyclo-DNA (tcDNA), locked nucleic acid (LNA), peptide nucleic acid (PNA) and phosphorodiamidate morpholino oligomer (PMO). Owing to the high liver toxicity issues of LNAs, constrained methyl- and ethyl-bridges in constrained methoxyethyl (cMOE) and constrained ethyl (cEt) oligonucleotides are often utilised.5 However, PMOs and PNAs are less toxic. But, they are neutrally charged and exhibit poor cellular uptake, requiring a bio-conjugate or carrier for effective delivery.5
Emerging technologies: The latest and what's to come
Mesylphosphoramidate (MsPA) linkage
MsPA linkage is an alternative to phosphorothioate for RNaseH1-mediated mRNA degradation. Here, one of the non-bridging oxygen atoms is replaced with methanesulfonylamido group. Replacing a few phosphorothioates with MsPA in gapmer ASOs showed improved potency and reduced cytotoxicity leading to a longer duration of pharmacodynamic effect in mouse models. 10
Dual sugar modifications
Compared to 2′ mono-modifications, the utilisation of dual modifications has demonstrated a synergistic effect in improving nuclease stability and extending the silencing activity in siRNAs. Examples of these modifications include 2',4'- and 2',5'-modifications, 5'-E/Z-vinylphosphonate, and northern methanocarbacyclic (NMC) modifications. Interestingly, research suggests that even a minimal number of dual ribose modifications could be sufficient to achieve the maximum therapeutic effect. 11
Nucleobase derivatives
The incorporation of certain nucleobase derivatives, such as 5-hydroxycytosine, 2-thiothymine and 8-bromoguanine, has been demonstrated to significantly reduce hepatotoxicity in LNA gapmers. Importantly, this nucleobase-modification technology is not site-specific, allowing for the potential of multiple modifications to synergistically decrease ASO hepatotoxicity. 12 Similarly, Guanidine-bridged nucleic acid (GuNA), a novel 2',4'-bridged nucleic acid/locked nucleic acid, exhibited strong affinity for target RNA and superior nuclease resistance. GuNA ASO is tolerable and exhibits lower hepatotoxicity in comparison with the LNA ASO. 13
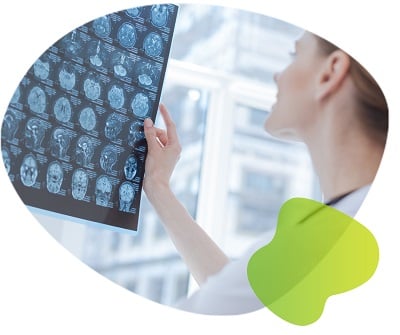
Besides GalNAc and lipid conjugates, a number of CPPs have been developed to enhance drug delivery of ASOs and siRNAs. CPPs comprise 6-30 amino acid residues that are potentially capable of intracellular penetration via endocytosis. Some examples include HIV-1 Tat peptide, MPG (N-Methylpurine DNA Glycosylase) derived from HIV glycoprotein-41 sequence, penetratin derived from the Drosophila antennapedia homeodomain, and artificial poly-arginine peptides. Amphipathic peptides such as MPG and penetratin can form stable non-covalent complexes with siRNA at certain molar and charge ratios which can be an alternative to covalent conjugation. 14 Furthermore, several conditionally activatable CPPs have been developed for selective delivery. One instance is the pH-sensitive transportan-CPP comprising lysine-to-histidine substitutions that can enter cells under acidic conditions, such as the tumour microenvironment. 15
Structural siRNA modifications
To increase the circulation time and biodistribution of siRNA, several structural modifications have been explored in pre-clinical models. Some of the promising approaches include circular siRNA, linear multimeric siRNA, branched multimeric siRNAs and Dicer Substrate.16 Experiments show that combining structural siRNA modification with rational chemical modification design will improve biodistribution and improve therapeutic properties.
Advanced solutions for ASO and siRNA synthesis
At LGC Biosearch TechnologiesTM, we're dedicated to advancing cutting-edge nucleic acid therapeutics for better patient outcomes. We upgrade our chemical modification toolbox regularly to keep pace with the latest technical developments and provide you with the most advanced tools for improving the stability, cell delivery, and efficacy of your ASO and siRNA therapeutics. More than 100 drugs, approved or in development, are made using at least one of our reagents.
Partnering with Biosearch Technologies means leveraging our technical expertise and our extensive product portfolio of oligonucleotide modifications, solid supports and reagents to help you stay ahead in this rapidly evolving field.
Partnering with Biosearch Technologies means leveraging our technical expertise and our extensive product portfolio of oligonucleotide modifications, solid supports and reagents to help you stay ahead in this rapidly evolving field.
Related content
- Nucleic acid therapeutics for next-generation disease management
- Overcoming manufacturing challenges associated with nucleic acid therapeutics
- Which controlled pore glass is right for your therapeutic application?
- A knack for getting to the liver: GalNAc enables liver-targeted oligonucleotide therapeutics
References
- Egli M, Manoharan M. Chemistry, structure and function of approved oligonucleotide therapeutics. Nucleic Acids Res. 2023;51(6):2529-2573. doi:10.1093/nar/gkad067
- Moumné L, Marie AC, Crouvezier N. Oligonucleotide Therapeutics: From Discovery and Development to Patentability. Pharmaceutics. 2022;14(2):260. doi:10.3390/pharmaceutics14020260
- Thakur S, Sinhari A, Jain P, Jadhav HR. A perspective on oligonucleotide therapy: Approaches to patient customization. Front Pharmacol. 2022;13:1006304. doi:10.3389/fphar.2022.1006304
- Crooke ST, Witztum JL, Bennett CF, Baker BF. RNA-Targeted Therapeutics. Cell Metab. 2018;27(4):714-739. doi:10.1016/j.cmet.2018.03.004
- Xiong, H., Veedu, R. N., & Diermeier, S. D. (2021). Recent Advances in Oligonucleotide Therapeutics in Oncology. International Journal of Molecular Sciences, 22(7). https://doi.org/10.3390/ijms22073295
- Alshaer W, Zureigat H, Al Karaki A, et al. siRNA: Mechanism of action, challenges, and therapeutic approaches [published correction appears in Eur J Pharmacol. 2022 Feb 5;916:174741]. Eur J Pharmacol. 2021;905:174178. doi:10.1016/j.ejphar.2021.174178
- Hu B, Zhong L, Weng Y, et al. Therapeutic siRNA: state of the art. Signal Transduct Target Ther. 2020;5(1):101. Published 2020 Jun 19. doi:10.1038/s41392-020-0207-x
- Thazha P, Bhat B. 2-Modified Oligonucleotides for Antisense Therapeutics. Current Topics in Medicinal Chemistry, 2007, 7(7), 641–649. doi:10.2174/156802607780487713
- Lim KRQ, Yokota T. Invention and Early History of Gapmers. Methods Mol Biol. 2020;2176:3-19. doi:10.1007/978-1-0716-0771-8_1
- Anderson BA, Freestone GC, Low A, et al. Towards next generation antisense oligonucleotides: mesylphosphoramidate modification improves therapeutic index and duration of effect of gapmer antisense oligonucleotides. Nucleic Acids Res. 2021;49(16):9026-9041. doi:10.1093/nar/gkab718
- Gangopadhyay S, Gore KR. Advances in siRNA therapeutics and synergistic effect on siRNA activity using emerging dual ribose modifications. RNA Biol. 2022;19(1):452-467. doi:10.1080/15476286.2022.2052641
- Yoshida T, Morihiro K, Naito Y, et al. Identification of nucleobase chemical modifications that reduce the hepatotoxicity of gapmer antisense oligonucleotides. Nucleic Acids Res. 2022;50(13):7224-7234. doi:10.1093/nar/gkac562
- Sasaki T, Hirakawa Y, Yamairi F, et al. Altered Biodistribution and Hepatic Safety Profile of a Gapmer Antisense Oligonucleotide Bearing Guanidine-Bridged Nucleic Acids. Nucleic Acid Ther. 2022;32(3):177-184. doi:10.1089/nat.2021.0034
- Singh T, Murthy ASN, Yang HJ, Im J. Versatility of cell-penetrating peptides for intracellular delivery of siRNA. Drug Deliv. 2018;25(1):1996-2006. doi:10.1080/10717544.2018.1543366
- Tarn WY, Cheng Y, Ko SH, Huang LM. Antisense Oligonucleotide-Based Therapy of Viral Infections. Pharmaceutics. 2021;13(12):2015. Published 2021 Nov 26. doi:10.3390/pharmaceutics13122015
- Chernikov IV, Ponomareva UA, Chernolovskaya EL. Structural Modifications of siRNA Improve Its Performance In Vivo. Int J Mol Sci. 2023;24(2):956. Published 2023 Jan 4. doi:10.3390/ijms24020956